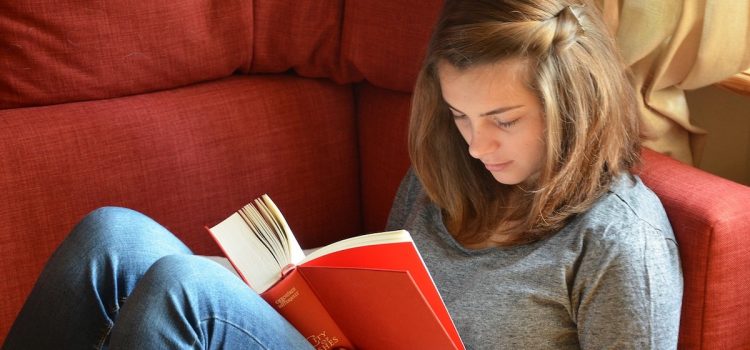
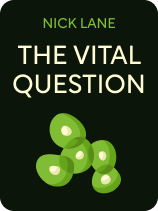
This article is an excerpt from the Shortform book guide to "The Vital Question" by Nick Lane. Shortform has the world's best summaries and analyses of books you should be reading.
Like this article? Sign up for a free trial here.
Why does life work the way it does? Are life’s features the product of random genetic mutations, or did organisms have to evolve in a certain way to overcome specific physical and chemical challenges?
In The Vital Question, Nick Lane argues that genetics can’t give us the answers by itself. To explain the origin of life, we have to understand how cells make use of energy and what environmental conditions gave rise to the molecular power plants found in every living thing.
Continue reading for an overview of this book that seeks to answer some of life’s biggest questions.
Overview of The Vital Question by Nick Lane
Biology has a big, unanswered question: “Why does life on Earth work the way it does?” Are all of life’s characteristic features the product of random evolutionary mutations, or did organisms need to evolve a certain way to meet specific physical and chemical challenges that were present at the dawn of life on this planet? Can the study of genetics and natural selection explain all of life’s mysterious quirks, or do we have to look deeper into the machinery of life to explain why certain peculiar properties are shared by every living thing on a microcellular level?
In his 2015 book The Vital Question, Nick Lane argues that genetics alone is insufficient to explain why living cells function in the specific way they do. He says that, to explain the origin of life, we have to understand how cells process energy and the physical conditions that gave rise to the peculiar mechanisms that all life uses to power itself. Answering how that process arose also gives us clues as to why life remained stunted in bacterial forms for two billion years before suddenly exploding into the myriad species that comprise the multicellular world.
Lane is a biochemist at University College London and the author of several books on molecular biology, including Oxygen and Power, Sex, Suicide. His 2009 book Life Ascending won the Royal Society Prize for Science Books. Lane has also been awarded the Michael Faraday Prize for his work in science communication and the Biochemical Society Award for his work in biology.
We’ll summarize the biochemical process by which living cells harness energy and how that process may have evolved in hydrothermal vents on the very young Earth. We’ll then describe why Lane believes all complex life may have emerged from a single, fortuitous instance of symbiosis between two different single-celled creatures with strikingly different properties.
How Life Works
Before discussing the origin of life, it’s important to think about what life is. Lane says that in its simplest form, life is a process by which matter self-organizes into complex, self-replicating molecules—a process that can only sustain itself with a continual flow of energy. That energy allows living systems to avoid decomposing long enough to reproduce, creating an internal electrical voltage to power microscopic molecular machines that use carbon atoms as their basic building blocks.
In our everyday experience, disorganized matter doesn’t spontaneously arrange itself into complex forms, any more than a pile of bricks can turn into a house without outside help. Yet, at first glance, it seems that life does this, especially when it comes to its origin. Not only can it seem unlikely that organization can arise from chaos, but doing so might be viewed as violating the laws of thermodynamics—which is why those laws must be clearly understood.
The second law of thermodynamics states that in any given system, entropy—the amount of chaos and disorder—will increase over time. However, living things seem to contradict this by growing, reproducing, and evolving into ever more complex, well-ordered systems of biochemical processes and molecular machines that have functioned for billions of years. Lane points out that this doesn’t violate the laws of physics. Life only decreases entropy in the short term, and it does so by increasing entropy around it—consuming its surrounding environment for fuel while expelling disorganized matter and energy as waste and heat.
This constant flow of energy from the outside world enables the order and complexity of life. What’s striking to Lane is the roundabout method life takes to facilitate this flow. In every living cell, an electrical voltage across a narrow membrane drives the production of a molecule called adenosine triphosphate (ATP), the fundamental unit of fuel that life uses. A typical cell “burns” ATP at a rate of 10 million molecules per second, and that ATP must be constantly replenished via cellular respiration—in which oxygen reacts with the chemical building blocks cells take from food, including carbon, nitrogen, hydrogen, and phosphorus.
The Engine of the Cell
Lane explains that within our cells are microscopic power plants known as mitochondria. Inside the mitochondria, various proteins rip hydrogen atoms out of food molecules, separating their protons and sending their electrons down a chain of oxygen atoms like a current down a wire. Each electron stop along the oxygen chain triggers a piece of protein machinery that shuttles a free proton across the inner mitochondrial membrane. The buildup of protons (and thus a positive charge) on one side of the membrane creates a strong electrical voltage, proportional in strength to a blast of lightning across the mitochondria’s infinitesimal space.
This electrochemical gradient powers the creation of ATP. What Lane finds particularly odd is that ATP synthesis isn’t a chemical reaction. It’s a physical construction process of atoms being assembled into molecules by protein machines running on electrical power. There’s no obvious evolutionary reasoning behind how microscopic yet powerful electrochemical gradients could have developed through random genetic trial and error, and yet these gradients are found in all life, even simple bacteria without mitochondria. The fact that they’re shared by all living beings implies that electrochemical gradients were a feature of life from the very beginning.
For Lane, life’s underlying chemical reaction is easy to understand. At its most basic, life runs on the transfer of electrons from hydrogen to carbon dioxide, both of which are common throughout the universe. Hydrogen and oxygen are soluble, stable, and ideal as donors and receptors of electrons, whereas the carbon in carbon dioxide is a perfect building block capable of forming long and complex molecular chains such as proteins, lipids, and even DNA.
Nevertheless, when Lane considers the commonalities among the three different branches of life—single-celled bacteria; their cousins, the archaea; and complex, multicellular eukaryotes such as plants, animals, slime molds, and ourselves—he finds vast differences in many of the most basic functions of life, even in the way DNA reproduces. From this, he deduces that our remote common ancestor, the life form from which all other life descended, was barely a cell at all in the common sense.
The First Cells
Evolutionary biology has a “chicken or egg” problem. The question is which came first—self-replicating molecules such as DNA or the organic structures of the cell within which those molecules thrive. Lane argues that researchers have ignored this problem by focusing exclusively on the lineage of the DNA molecule, but the origins of life can’t be fully understood without also discussing where it began and what sort of environmental conditions gave rise to the first life on Earth. The fossil record and genetic evidence give some clues to the path on which life developed, but there are gaps in our knowledge that scientists can’t fill via direct observation.
Consider what our world was like four billion years ago. The chemical makeup of ancient zircon crystals shows that the young Earth was temperate and wet, but the atmosphere was primarily carbon dioxide, nitrogen, and water vapor. There wasn’t any free oxygen yet, since that’s a byproduct of organic respiration. The earliest geological evidence of the chemistry of life dates back 3.8 billion years, but the first microfossils of single-celled organisms don’t appear until 300 million years later. Lane admits that much of this is still up to debate because, that far back in prehistory, the dividing line between biochemistry and geochemistry is fuzzy since there are some naturally occurring chemical reactions that can be mistaken for signs of simple life.
Genetic History
Whereas geologists look to the fossil record and traces of ancient chemical reactions to study the origins of life, biologists chart life’s ancient lineage by comparing the genomes of different modern species, but here we run into a different problem. Genes reveal the history of evolution via commonalities in species’ DNA.
However, Lane explains that, for most single-celled life, this doesn’t work because of a process called lateral gene transfer. In essence, microbes swap DNA with each other, creating hybrids and Frankenstein’s monsters on the fly. This makes it impossible to use shared DNA to trace the history of bacterial species since any DNA within a bacterium could have come from its parent or a completely different neighbor.
What microbial DNA shows for certain is that deep in prehistory, single-celled organisms split into two separate domains of life—bacteria and the more recently discovered archaea, simple life forms that look like bacteria but are very different on a genetic and molecular level. Since archaea share some similarities with multicellular life on the microscopic level, it was first believed that they were either an offshoot or a “missing link” in our evolutionary line, though Lane argues that the story is actually more complex.
How to Make a Cell
To deduce how life began, biologists like Lane have to reverse-engineer our primordial single-celled ancestors. Working backward through time, it’s possible to hypothesize the traits of the very first microbial life. Dubbed the last universal common ancestor, the first cell contained only the biological features found in all living cells today, but not characteristics that evolved later—such as different species’ internal chemistry or the different ways that cells divide. Lane discusses the minimum structural requirements a living cell needs to survive and reproduce, the environmental factors that must have been present for life to arise from nonliving matter, and the sequence of events that may have taken place to ignite the first spark of life.
What a Cell Needs
The most easily identifiable parts of a cell are the self-replicating molecules that encode its chemical blueprint—DNA and RNA. The next requirement that makes a cell a “cell” is a physical structure to define its boundaries. Beyond that, a cell needs a steady supply of food and energy, as well as some kind of catalyst for metabolic reactions (which break the food apart into building blocks and ATP). Finally, the cell needs a way to eject the waste products of its metabolism so that they don’t clog the cell with useless matter. Lane says all these features had to be there from the start, which returns us to the question of which came first—the structure of the cell or the DNA blueprints for how to build one.
Lane suggests that the answer to the riddle is that life arose in an environment where many of its necessary components already existed in a nonliving form. We know that matter can spontaneously organize itself, given enough energy input.
Cells Without Borders
While the same basic organic compounds are common to all known forms of life, one trait that isn’t shared between bacteria and archaea is the structure of the outer cell wall. Both have them, but both have significant differences on the molecular level—differences strong enough to suggest that the two domains’ cell wall structures developed independently from each other in a case of parallel evolution. From this, Lane concludes that the original cell didn’t have an organic cell wall at all—the cell wall evolved later in the evolutionary time frame. Since the first cell had to have some kind of boundary, that boundary must have been inorganic, perhaps even geological in nature, which gives our first clue as to where life may have formed.
The second clue to where life may have formed is the electrochemical membrane gradient that’s used to power all living cells. The original cell must have been born in a place where such gradients can occur naturally. Finally, there’s the requirement for the cell to metabolize food into the molecular building blocks it needs to grow and reproduce. In modern cells, the flow of food and waste into and out of the cell is guided by complex, specialized proteins that have evolved over eons. Lane argues that before life began, those specialized proteins couldn’t have evolved yet, so something else must have directed that flow—perhaps a stream of heated organic compounds funneled through a constricted passage.
Where Life Began
Because of these clues, Lane believes that a certain chain of events took place that created the housings for the first living cells. First, inside a porous geologic formation with microscopic chambers the size of tiny cells, natural electrical gradients combined carbon and other common elements into organic molecules. Those molecules clustered in the formation’s nooks and crannies, where a steady stream of heat prompted them to self-organize into even more complex patterns. Eventually, some of these molecules coalesced into chains that are capable of copying themselves, while others formed the basis of internal cellular structures. But where in the world could this sequence of events have taken place?
Lane writes that taking into account the first living cells’ chemical and geophysical requirements narrows the field of possible locations where life could have begun to a single, most likely candidate: alkaline hydrothermal vents at the bottom of Earth’s primordial ocean. Lane describes the nature of these hydrothermal vents, why they were ideal for the development of life, and a scenario by which life may have evolved the ability to break free from these vents and survive in the open ocean.
Alkaline hydrothermal vents are different from the more commonly known “black smoker” underwater volcanic vents that exist along oceanic ridges where continental plates are pulling apart. Alkaline vents are farther from the rift, but where molten rock from the mantle is still relatively close to the Earth’s crust. Seawater filters down through the crust, where it reacts with the mantle and spews back upward, bringing a payload of hydrogen gas and minerals from beneath the Earth’s surface. These vents create porous towers of rock that are rushing with heated water rich in chemicals—conditions that are perfect for organic compounds.
Life in the Vents
According to Lane, the micropores in these vents were the ideal cell-like structures in which the chemical precursors of life could collect. The material found in the walls of these vents, such as iron sulfide and iron hydroxide, acted as inorganic catalysts for the basic metabolic reaction of life—the conversion of hydrogen and carbon dioxide into a variety of organic molecules until finally being expelled as methane. The flow of warm, chemically saturated water provided all the energy and fuel this burgeoning ecosystem needed, while also providing a motive force to flush away the buildup of waste from the first organic reactions.
Lane goes on to point out that oceanic chemistry was different four billion years ago, and this was significant to the formation of life. The seas of that time had no dissolved oxygen, but they were rife with carbon dioxide, meaning they were far more acidic than today. The water emerging from these vents, however, was strongly alkaline. The fatty acids created by the vents’ ongoing chemistry congealed into a membrane-like barrier between the vents’ porous interior and the seas outside. The iron that coated the walls of the vents conducted protons from the acidic (positively charged) ocean to the alkaline (negatively charged) water in the vents’ pores, creating the electrochemical gradient that still powers all living cells today.
In other words, the physical properties of alkaline vents provided all the necessary pieces for living cells to develop within them. All that was needed now for life to start was for the proteins and organic molecules inside to organize into patterns that could store information and replicate themselves. Once that happened, life was ready to emerge, spreading throughout the alkaline pores in life’s original hydrothermal home.
Life Escapes the Vent
If alkaline vents were the perfect environment for the earliest form of life on Earth, what was it that pushed that life to leave its home and explore the rest of the planet? Lane says that the answer lies in how cells make their own energy. Modern cells use an electrical voltage across a membrane to power the creation of the ATP molecule, a voltage that is created by tiny protein machines moving charged ions from one side of the membrane to another. However, inside an alkaline vent, there was no need for those proteins to evolve. The naturally conductive iron inside the vent provided all the electrical pathways that were needed—so long as the charge was from hydrogen ions.
Lane and his colleagues have discovered certain enzymes that pump sodium ions across cell membranes in addition to hydrogen. These enzymes provide an evolutionary kick because sodium ions yield more power than hydrogen, and being able to pump them out of the cell reduces the buildup of sodium inside. However, this only works if the cell has a thicker, less permeable membrane to keep the excess sodium out. Lane proposes that the evolution of sodium-ion power prompted the evolution of stronger cell walls and freed life’s ancestors from the natural conductors found in alkaline vents. Once the cell wall and ATP-building enzymes were in place, our forebears floated free of their vents and into the ocean at large.
Lane suggests the evolutionary leap to sodium-ion power actually happened two different times, among two separate populations of cells that sprang from our last universal common ancestor. This division gave birth to the two separate domains of single-celled life: bacteria and archaea. Scientists believe this because those two separate domains found different solutions to the same evolutionary problems. Their cellular membranes, enzyme pumping machines, and even the way they metabolize carbon all function differently enough to suggest that they took separate evolutionary paths to break free from the oceanic vents. The stage was set for life to flourish, but the next giant leap in its development wouldn’t occur for two billion years.
The First Complex Cells
The event that prompted the explosion of complex life from simpler, single-celled forms has long been shrouded in mystery. Lane proposes that eukaryotes—the domain of life that includes everything more advanced than bacteria and archaea—arose from a single, unlikely symbiosis between two very different single-celled creatures that unlocked the limits on how cells use energy and undammed the flow of evolutionary progress. Lane explains what distinguishes eukaryotic cells, the idea of evolution by symbiosis, and the energy problem that holds bacteria and archaea back while propelling eukaryotic life to ever greater organization and complexity, eventually evolving into the plant and animal kingdoms.
What defines eukaryotic life is that the cell’s DNA is contained within a protective nucleus, whereas in archaea and bacteria, the DNA is attached to the outer cell wall right next to the enzymes that produce ATP. Not every eukaryote is multicellular, but they all dwarf bacteria and archaea in terms of the size of their genome and the complexity of their molecular machines. Eukaryotes are full of mitochondria that generate the relatively enormous amount of power eukaryotic cells need to enact the properties of their multitude of genes.
To be sure, bacteria and archaea are incredibly varied in terms of biochemistry, but they don’t come close to eukaryotes in terms of size and complexity. What’s more, bacteria and archaea haven’t evolved cellular complexity in four billion years. Lane suggests that there’s something physically different about eukaryotic cells that allows them the structural complexity that bacteria and archaea can’t achieve. What puzzles biologists is that they’ve found no “missing link” between simple, single-celled organisms and eukaryotes as if the first eukaryotic cell sprang into existence fully formed.
The Great Merger
An analysis of eukaryotic genes further confuses the issue of complex life’s evolutionary heritage. Eukaryotes share a third of their genome with single-celled life, but 75% of those genes come from bacteria while the rest come from archaea, as if eukaryotes somehow descended from both domains of simple organisms. Lane says that this is evidence that the first complex cell was a hybrid of a bacterium and an archaeon that somehow grafted themselves together. Because this shared genome is common to all eukaryotes, biologists are forced to conclude that this hybridization only happened once in four billion years, or at least only once that was successful and survived.
The process by which such grafting can occur is called endosymbiosis, in which one cell is physically engulfed by another, but instead of being consumed, the two cells become a larger, combined organism. The evidence that this happened lies in our mitochondria, which have a completely separate genome from the nucleus of the cell. Mitochondria are, in fact, an evolutionary remnant of that first coupling.
Once, long ago, an archaeon absorbed a bacterium in such a way that allowed the bacterium and its descendants to flourish inside the archaeal host cell. Lane explains that the host stripped the bacteria’s DNA, adding it to its own repository, while leaving the bacteria just enough genes to produce ATP and fuel the host cell’s growth. As evidenced by the mitochondrial genome, our modern mitochondria are the descendants of those bacteria, reduced to the status of power batteries that are fed and protected by the larger eukaryotic cell.
The Energy Problem
The fact that the initial archaea-bacteria chimera thrived suggests that its odd symbiosis provided the first eukaryotes with an evolutionary advantage. As it was with the original genesis of life and the adaptation that let it leave its ocean-vent nest, the development of complex cellular structures revolved around a new way to harness energy. Lane describes the energy restrictions that have kept bacteria and archaea from achieving complexity for billions of years, while the novel approach that eukaryotes took to overcome that energy barrier not only allowed for greater structure and complexity but actually drove genetic development.
One fundamental difference between eukaryotes and simpler life forms is that because of their mitochondria, eukaryotes have vastly more energy available for use per gene. That energy goes into constructing proteins based on DNA’s genetic designs. Without mitochondria to fuel them, bacteria and archaea have to rely on the ATP their genes produce along their outer cell wall. Lane says this encourages them not to grow bigger because as a bacterial cell’s volume increases, its available energy only grows proportional to its increase in surface area. A large cell is, therefore, less energy efficient and would be outcompeted by its leaner, meaner cousins.
Because of the efficiency problem, Lane argues that there’s no evolutionary reason for archaea and bacteria to grow in size and complexity. By adapting their inner metabolic processes, single-celled organisms have adapted themselves to flourish in every corner of the ecosphere, including the Earth’s most extreme environments. There’s no need for more complexity or larger genome size, so evolution has instead selected for bacteria and archaea that are slimmed down to the basic needs of survival.
During the endosymbiosis event that launched the beginning of eukaryotic life, the evolutionary pressure for lean, efficient cells got flipped on its head. The newly absorbed mitochondrial bacteria provided far more energy than the archaeal host cell needed. Lane suggests that rather than being drowned with ATP molecules, the host cell had to find something to do with all that excess energy. Its solution came from the extra DNA it absorbed and repurposed from its bacterial symbiotes. The flood of new energy demanded it be spent, and the only way for eukaryotes to survive was to open the possibilities of complex cell structures, charting new frontiers for evolution to explore.
The Path of Evolution
Because of the lack of intermediary steps on the evolutionary path to eukaryotes, it’s not easy to reconstruct the steps from that first symbiosis to the modern complex cell. But just as Lane reverse-engineered the properties of the original cell, he is able to do the same for eukaryotes, tracing our ancestors’ rapid evolution from a chimeric hybrid to a new branch of life. The clues he draws upon are the introns—so-called “junk DNA”—that populate eukaryotic genomes but not that of bacteria and archaea. He shows that a sudden flood of such introns, stripped from bacteria in the eukaryotic host cell, populated the newly forming species and caused the evolution of the nucleus’s wall.
One thing that’s certain is that the nucleus evolved after endosymbiosis. Lane explains that we know this because a similar structure doesn’t exist in any species of archaea or bacteria. Also, because all eukaryotes have a nucleus, we know that it evolved relatively quickly—before eukaryotes had a chance to evolve into separate species. Evolution favors stability, and new adaptations only appear when absolutely needed for survival. Therefore, the merger of archaea and bacteria created an unstable internal situation that natural selection had to address quickly.
The instability of the original endosymbiotic merger is reflected in the chaotic state of eukaryotic DNA. Between useful strands of genetic information, the eukaryotic genome is full of dead lines of code—known as introns—that don’t produce any proteins at all. Lane traces the lineage of these introns to genetic parasites that inhabit bacterial DNA. These parasites aren’t living things themselves but are self-replicating bits of DNA that hitchhike inside bacteria and spread through lateral gene transfer. Bacteria and archaea have internal defenses such as enzymes that act as genetic pest control to stop these parasites and keep their numbers down.
Lane hypothesizes that something different happened during endosymbiosis. As the absorbed bacteria lost DNA to the archaeal host cell, it also released a flood of genetic parasites, and because they were parasites from bacterial DNA, the archaeal host cell had no defenses. These parasites, the precursors of introns, flooded the burgeoning eukaryotic genome and began to make use of excess ATP to generate a torrent of unusable proteins. Since the cell had ample energy, this wasn’t a problem until those proteins began to clutter the insides of the cell. Only then did evolution come up with a way to turn the introns off.
The Nucleus Is Born
According to Lane’s proposal, our first eukaryotic ancestors evolved a way to shut down our introns by walling them off behind a membrane, the one that defines the outline of the nucleus. This membrane interrupts the process of expressing intron genes as useless protein strands while allowing the genes that should be active to carry out the tasks they’re assigned for. The nuclear membrane is able to do this because it’s based on bacterial DNA and can therefore defend against parasitic bacterial genetic code. While our ancestral bacteria became mitochondria, our ancestral archaea appropriated bacterial traits, such as the kind of protective membrane that could deactivate the introns blocking our single-celled forebears’ evolution.
With the formation of the nucleus and the birth of mitochondria, the evolutionary floodgates of eukaryotes were opened. The powerhouse of the cell and the flexibility of a large genome enabled the many evolutionary wonders of the natural world. Gone are the days when bacteria and archaea were the preeminent forms of life on the planet. All this, Lane says, took place because of a single instance of symbiosis that’s only happened once in the history of our world.
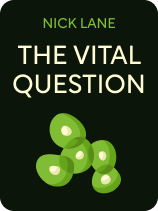
———End of Preview———
Like what you just read? Read the rest of the world's best book summary and analysis of Nick Lane's "The Vital Question" at Shortform.
Here's what you'll find in our full The Vital Question summary:
- Why genetics alone aren't enough to explain why cells function as they do
- A theory on where all complex life originated from
- An explanation of how cells work and are made